Jet measurements at the LHC
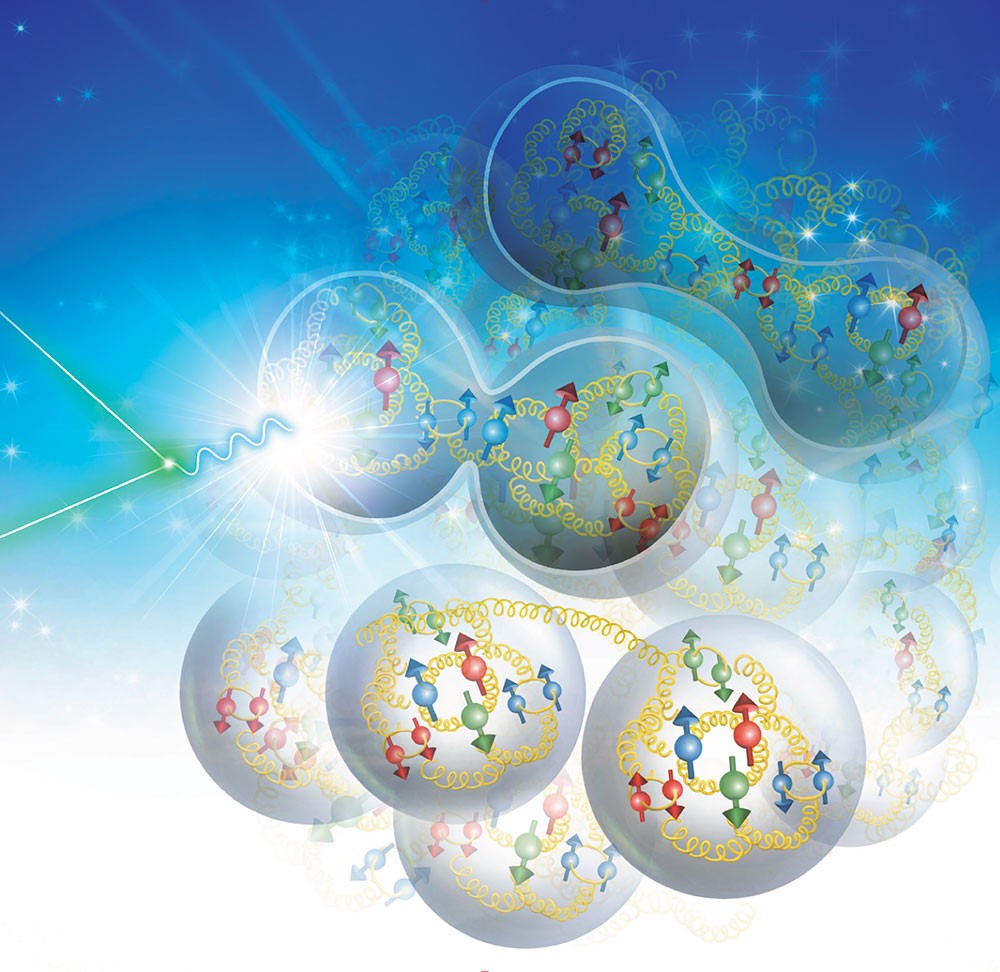
At the Large Hadron Collider, beams of protons or nuclei are accelerated nearly to the speed of light – traveling around the 27-kilometer accelerator ring over ten thousand times per second. The beams are then smashed into each other at the center of five-story-tall detectors, which map the trajectories of individual particles that emanate from the collisions. The purpose of all of this is to piece together the microscopic dynamics of the collision from these detected final-state particles – and look for something interesting. What do we mean by interesting? To some people that means searching for dark matter or for clues to the physical laws that govern energies far above our own. To others, it means understanding how complex behavior emerges from fundamental laws – such as in the strongest force of nature, known as quantum chromodynamics, or QCD.
QCD is one of the most prolific, yet most poorly understood, forces in nature. It is QCD that binds quarks and gluons into protons, overcoming the enormous electric repulsion of nearby quarks to confine them into eternal, swirling agglomerations of particles. These “simple” bound states, sketched in the image above, are not simple at all – quarks and gluons pop in an out of existence, interacting with each other in complicated substructures and superstructures. Understanding how these complex dynamics result in a simple, stable bound proton is one of the biggest open questions in physics: protons form the basis for stars and atoms, the very building blocks of life as we know it.
The basic equation describing all interactions of the strong force is already known – it is a part of the Standard Model of Particle Physics. However, there is a minor problem: we do not know how to solve the equation! This is because even the simplest QCD system is a complex, large-scale system – it is intrinsically high-dimensional due to the nature of quantum field theory. This presents a profoundly subtle situation: we know the “fundamental” law, yet we are unable to explain its most important consequence. This principle – the emergence of complex behaviors from large-scale systems – is at the heart of QCD (it is this same property that is driving some of the most exciting advances in computation, including both artificial intelligence and quantum computing).
There are two methods that have been developed to approximate the solution for certain limits of the equation: perturbative QCD and lattice QCD. These methods have enabled tremendous success in calculating high-energy scattering rates and static properties of nuclear matter such as the proton, yet they leave a fundamental question open: What are the dynamics of confinement, which traps quarks and gluons into the nucleons that populate our universe in the first place? My research focuses on two approaches to this question – the first by studying the limits of perturbative QCD in proton-proton collisions, and the second by studying the high-temperature phase of QCD known as the quark-gluon plasma.
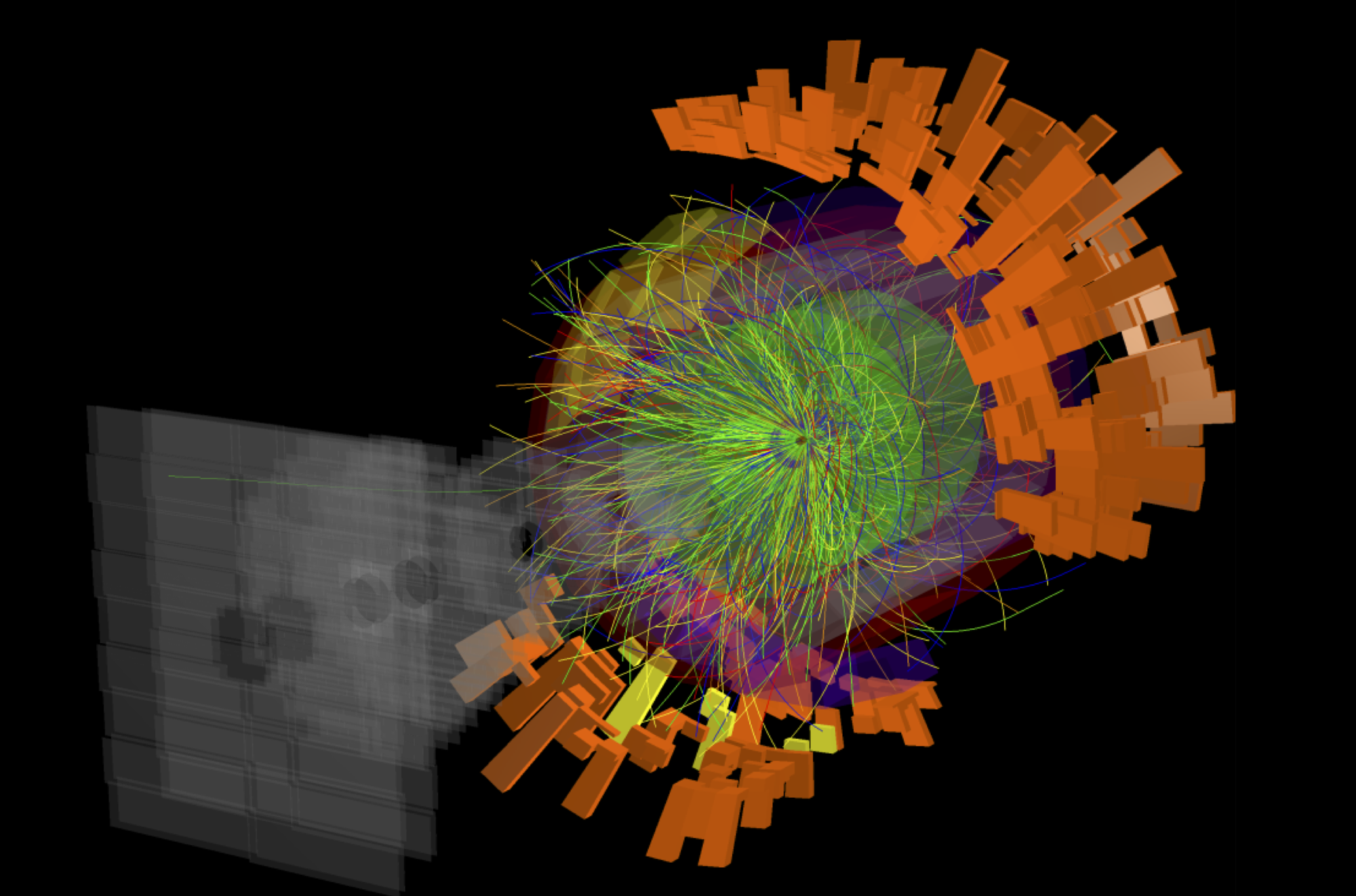
Jets in proton-proton collisions
In proton-proton collisions, two quarks or gluons will sometimes exchange a particularly large momentum and produce a high-energy outgoing quark or gluon. This high-energy quark or gluon will fragment into a collimated shower of particles known as a jet. Since the momentum exchange is large, one can use perturbative techniques to predict properties of these jets, such as the rate at which they are produced, and the pattern of the particles within the jet, known as jet substructure. Jets therefore enable direct tests of perturbative QCD calculations, which are a cornerstone of searches for physics beyond the Standard Model. But jets also elucidate the extent to which perturbative methods can describe the fragmentation of quarks and gluons into hadrons – a key ingredient to studying confinement.
My research explores the perturbative to non-perturbative transition, testing high-order perturbative calculations and non-perturbative scaling laws [1] [2] [3] [4] [5]. These measurements not only help build a precise understanding of the QCD background for searches for new physics but also lay groundwork for understanding the hadronization process at the future Electron-Ion Collider.
Jets in the quark-gluon plasma
If, instead of colliding protons together, we collide heavy nuclei such as lead, the energy density of the collision is so high that it produces a short-lived droplet of an entirely different phase of QCD matter: the quark-gluon plasma. This is the hottest, densest form of matter known to humans, and occupied the early universe for most of its first few microseconds. At such high energy densities the interactions between quarks and gluons becomes weak due to asymptotic freedom – causing the protons and neutrons to melt into a deconfined sea of quarks and gluons. Despite the interactions being too weak to bind the quarks and gluons into nucleons, they remain sufficiently strong to form a strongly-coupled liquid. The microscopic structure of this liquid remains unknown, and the emergence of this strongly-coupled system from a quantum field theory is one of the major outstanding questions in QCD.
In order to investigate the fundamental degrees of freedom of the quark-gluon plasma, one needs to study it at a variety of length scales. While the QGP is too small and short-lived to be probed with traditional scattering beams, jets can be used as probes: when a jet is produced in a heavy-ion collision, it will traverse the hot, dense quark-gluon plasma, and its fragmentation pattern will become modified.
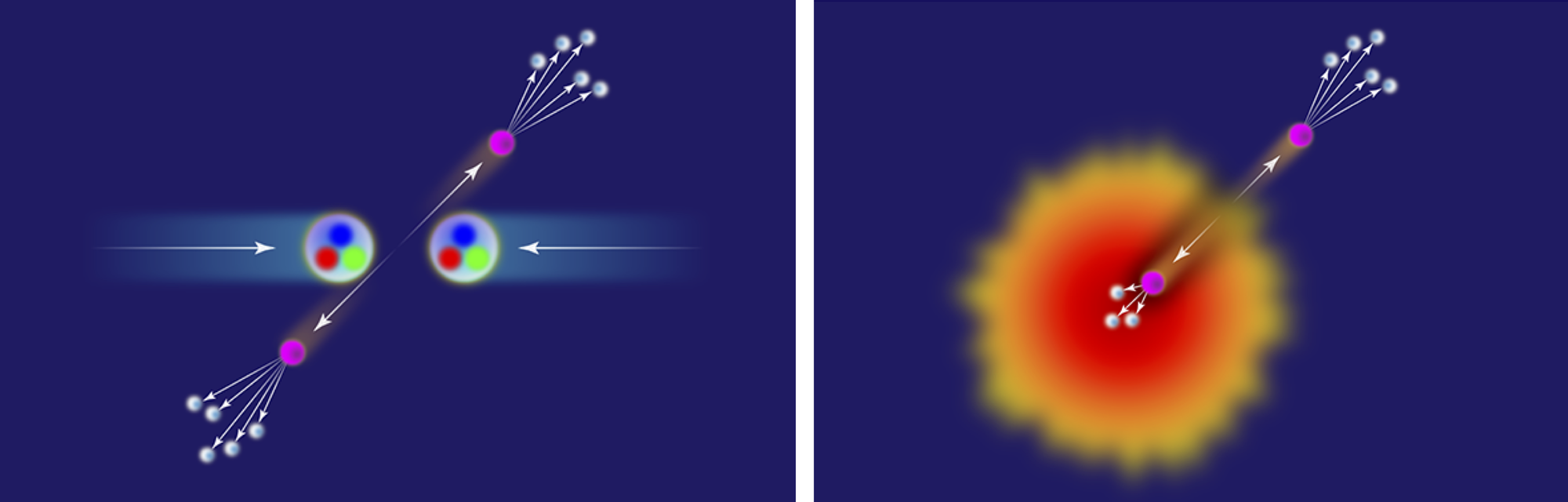
In my research, I have performed jet measurements in heavy-ion collisions of carefully designed jet properties that can be directly compared to theoretical calculations [1] [3] [6] [7] [8]. These measurements have shown that the energy of jets emerging through the quark-gluon plasma is, on average, lower than those that did not pass through the plasma [1]. Moreover, I found the first direct evidence of the modification of the angular structure of jets in heavy-ion collisions – placing the qualitatively new constraints on the microscopic structure of the quark-gluon plasma [6]. These measurements provide a new window into the emergent structure of QCD, which we delve further into in the next section using machine learning and Bayesian inference.